Feature
LIGO: Finally Poised to Catch Elusive Gravitational Waves?
After a seven-year upgrade project incorporating cutting-edge lasers and optics, the Advanced Laser Interferometer Gravitational-Wave Observatory could start directly detecting gravitational waves as early as next year.
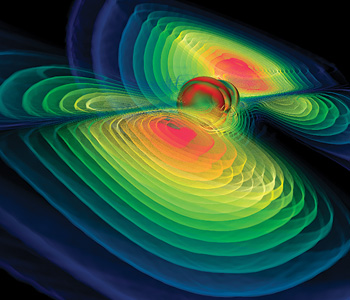
Computer simulation of a black hole collision. When two black holes merge, enormous amounts of energy are released in the form of gravitational waves. [Bernd Brügmann, University of Jena, Germany]
The initial Laser Interferometer Gravitational-Wave Observatory (LIGO) instruments began collecting data from the cosmos in 2002 and concluded operations in 2010. After years of research and development, work began in 2008 on Advanced LIGO, a major upgrade that will increase LIGO’s sensitivity; this project is scheduled for completion at the end of March 2015. Researchers hope that the Advanced LIGO project not only will make the first direct observations of gravitational waves in the coming years, adding weight to Albert Einstein’s nearly 100-year-old theory of general relativity, but also will open a window to the universe that astrophysicists and astronomers will use for many decades.
…Log in or become a member to view the full text of this article.
This article may be available for purchase via the search at Optica Publishing Group.
Optica Members get the full text of Optics & Photonics News, plus a variety of other member benefits.